Understanding the intricacies of cell division and how it sustains life
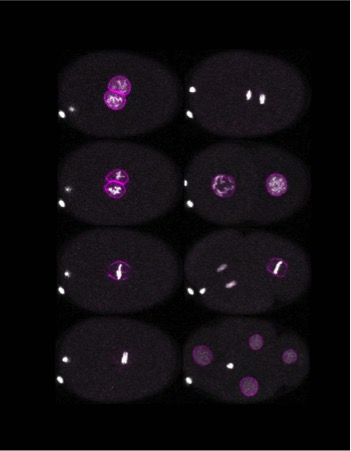
(Image courtesy: Sachin Kotak lab)
If you have flipped through a biology textbook, you’ve likely encountered the iconic image of a cell dividing into two identical offspring. Cells – the fundamental building blocks of life – keep every organism alive and thriving, from the smallest microbe to the largest mammal. Among the many processes that sustain life, cell division is perhaps one of the most essential.
Every day, around 300 billion cells divide in a human body. They replace old or damaged cells, support growth, and keep us alive. But how does a complex process run so seamlessly within a space as small as a single cell?
At its core, cell division is a series of precisely timed steps – the cell contents are first duplicated, then separated into two compartments and finally divided, resulting in the formation of two identical daughter cells.
“Imagine splitting a balloon into two without letting it pop,” says Saravanan Palani, Assistant Professor at the Department of Biochemistry. Before this split happens, cells undergo a preparatory phase called interphase which typically constitutes ~90% of the cell division cycle. During this stage, the cell duplicates its DNA and synthesises the proteins needed for division.
By the time the interphase ends, the cell’s DNA is wholly duplicated, ready to be part of a new cell. However, it still faces a significant challenge: the DNA is encased within the nucleus, a specialised compartment inside the cells. A successful division cycle requires the formation of two nuclei containing the DNA. “Different organisms handle this in unique ways,” explains Sachin Kotak, Associate Professor at the Department of Microbiology and Cell Biology. “Animal cells typically break down the nucleus entirely and reassemble it once the DNA is segregated, while some fungi manage division without dismantling the nucleus.” Once the two copies of DNA are separated into daughter nuclei, the whole cell divides into two daughter cells.
Protein players
Much of what we know about these processes is by studying critical molecular players, such as cyclins and cyclin-dependent kinases (CDKs). These proteins orchestrate various phases of the cell cycle. However, Sachin points out that there’s still much to learn, especially with the discovery of diverse proteins participating in cell division. “We understand the role of individual proteins by deleting that specific gene and observing the changes [in the cell]. But how these proteins communicate with one another to coordinate the cycle remains a mystery. That’s a major goal for our lab,” he says.
A crucial step during cell division is condensing the DNA. During interphase, the DNA is like a mass of tangled threads, like spaghetti. For division, this genetic material is compacted into tightly wound structures called chromosomes, where the DNA wraps around histones and other proteins. These chromosomes then align at the centre of the cell and are pulled apart into two sets, one for each daughter cell. This step relies heavily on the cytoskeleton – a dynamic network of protein fibres found in the cell.
“Even if you perfectly segregate the genome [DNA], it’s useless unless you also physically split the cell,” Sachin points out. The cytoskeleton, comprised of the proteins actin and tubulin, is critical for separating the daughter cells. Tubulin forms hollow, rod-like structures called microtubules, which act as scaffolding and transport highways within the cell. These microtubules attach to chromosomes and create a diamond-shaped mitotic spindle apparatus that aids in bringing the chromosomes to the centre of the cell through mechanical forces.
Sachin’s lab has worked intensively on the nuclear mitotic apparatus (NuMA) protein, also crucial for cell division. NuMA is present in the nucleus during the interphase. The team found that it localises asymmetrically at the cell membrane (present in the polar region and excluded from the equatorial region of the cell membrane) in the dividing cells. Beyond its role in spindle formation, Sachin’s team has uncovered how NuMA influences the positioning of the division plane. “We’ve found that membrane-localised NuMA plays a key role in forming the division furrow by confining the machinery involved in cytokinesis (division of cytoplasm) to the cell’s equator,” he says. It is at this region where the cell eventually pinches into two daughter cells.
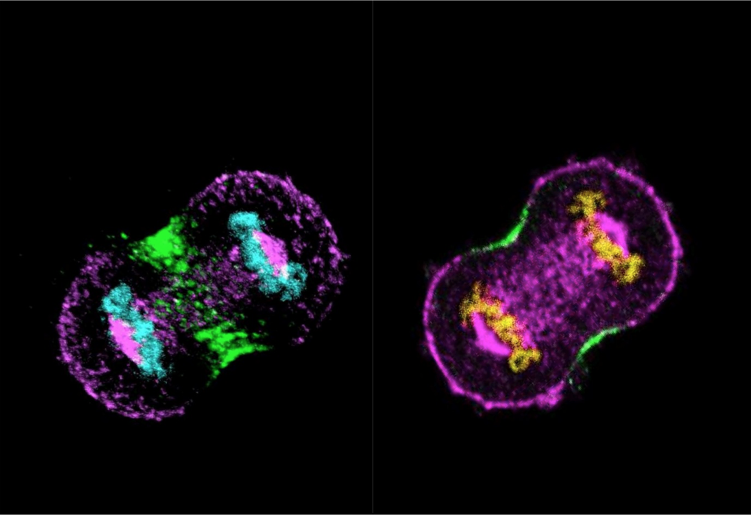
(Image courtesy: Sachin Kotak lab)
Crucial checkpoints
But how does the cell ensure that each step in this complex process happens in the correct order? “Different checkpoints regulate the cell cycle,” explains Saravanan. “If something goes wrong – like insufficient nutrients or incomplete DNA duplication – the cycle pauses until the issue is resolved; only then does the cell proceed.”
While checkpoints regulate most stages of division, there’s no known checkpoint regulating the physical separation of the cells, a stage known as cytokinesis. What happens when the cytoskeletal machinery fails during this critical step? “Failures during cytokinesis can lead to severe consequences like uncontrolled cell growth,” says Saravanan, whose lab studies this process. “Understanding these failures is important if we want to prevent or treat conditions such as cancer,” he adds.
At the end of the cell division cycle, cytokinesis leads to the formation of a physical barrier and separation of the daughter cells. The barrier is formed by pinching the cell membrane into the cell and requires a significant amount of mechanical force. Once again, the cytoskeleton is crucial for this step. “Actin filaments act as microscopic tracks on which molecular motors, like myosin, run. These motors generate the force needed to pull the membrane inward, facilitating membrane invagination,” Saravanan explains.
Lifesaving research
Today, we understand the intricacies of cell division in great detail. These studies could lead to better cancer therapies, more effective treatments for emerging pathogens, and even novel strategies to target harmful organisms while sparing healthy cells.
However, much of our understanding comes from conventional model organisms, like yeast (Saccharomyces cerevisiae and Schizosaccharomyces pombe), Caenorhabditis elegans, and Drosophila melanogaster which are adapted to the lab environment and amenable to genetic modifications.
While these systems have provided invaluable insights, other non-model organisms, often less studied and lacking extensive genetic tools, are now gaining attention for their potential to answer new questions. “Non-model organisms are essential for uncovering unique mechanisms,” says Sachin, who also explores cell cycle dynamics using human cells in culture.
Saravanan agrees, emphasising the urgency of studying the cell division machinery of non-model organisms to discover novel ways to target pathogenic microbes. “[For example], Cryptococcus neoformans, a fungal pathogen, is causing a silent health crisis due to its ability to attack immunocompromised patients. Yet we know very little about its cytoskeleton.” Understanding the cytoskeleton of this notorious fungus will pave the way for designing therapies to target this organism.
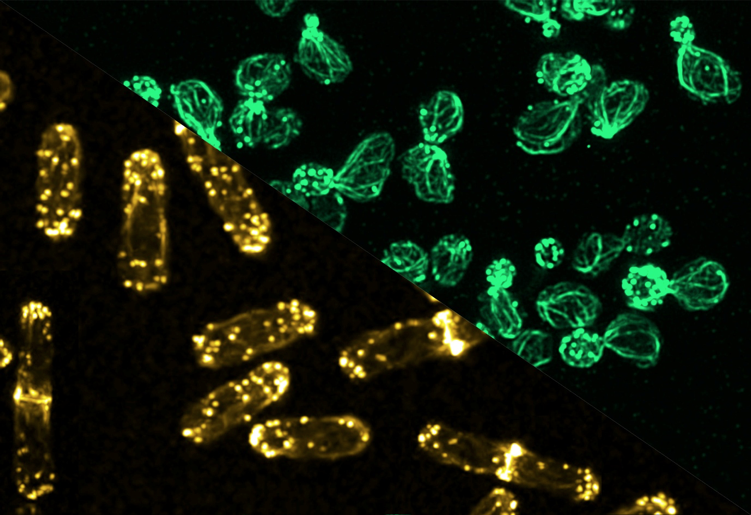